Plasmonic cavities are an effective tool for improving optical processes in nanoscale materials. Tightly confined optical fields at junctions between metal nanoparticles can lead to an increase in local light intensity by up to several orders of magnitude. This allows surface-enhanced Raman scattering, surface-enhanced infrared absorption, strong coupling and the Purcell effect - spontaneous enhancement of light emission.
In this case study from Andor, plasmonic cavities were used to enhance photoluminescence (PL) upconversion. This is a process in which light is emitted at a higher energy than an excitation laser. PL upconversion can generally occur due to two-photon excitation or also by extracting thermal energy from the material. This process can also lead to radiative cooling of the material, simply by illumination. PL upconversion occurs in many material platforms, including rare earth doped solids, semiconductor nanoparticles and 2D materials. Especially in 2D materials, upconversion is usually weak because of the lack of a combination of energy levels for excitation and emission. In our recent work1, we have activated the excitation channel for PL upconversion in single-layer WSe2 by coupling a near-field plasmonic cavity to a spin-forbidden dark exciton.
Assembly
We used a fully automated setup to characterize the process in which the photoluminescence of single-layer WSe2 is enhanced by plasmonic nanocavities. Photoluminescence spectra were recorded using an Andor Newton 970 BVF EMCCD camera attached to an Andor Kymera 328i spectrometer with a 150 l/mm grating. PL was excited and collected in a homemade dark-field optical microscope with automated nanopositioning stages, an optical camera, and excitation with two cw lasers (633 nm and 785 nm) (Fig. 1). Nanocavities were identified by their scattering pattern in the dark field using image recognition algorithms and centered on the measurement site. Time traces of PL spectra were recorded on each nanocavity to monitor PL stability and rule out damage. PL spectra were then recorded on each cavity with laser excitation at 633 nm and 785 nm. The two lasers were combined with a dichroic beam splitter, switched using a shutter, and the respective notch filters in front of the spectrometer were switched using a motorized filter slider(Thorlabs). All instruments were interfaced using a home-written nplab interface in Python (https://github.com/nanophotonics/nplab) using the Andor software development kit. This allowed subsequent PL spectra of hundreds of individual plasmonic cavities to be recorded.
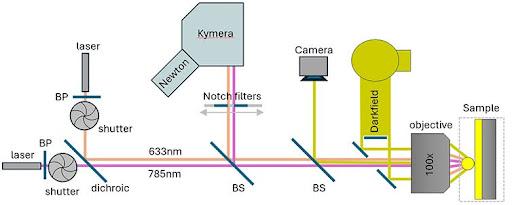
Figure 1: Schematic optical setup combining dark-field imaging with 633 nm and 785 nm laser excitation for optical interrogation of a single nanoparticle on a mirror.
Results
We used nanoparticle-on-mirror (NPoM) plasmonic cavities to enhance the light emission of 2D materials (Figure 2a). Gold nanoparticles were cast on a gold substrate, which was covered with exfoliated flakes of single-layer WSe2. In this geometry, the 2D material defines a nanometer gap between the nanoparticles and the Au mirror. The plasmonic nanoparticles act as optical antennas and confine the light to this nanometer gap, leading to a thousand-fold increase in local light intensity.
These cavities were localized through their dark-field scattering patterns, which are horseshoe-shaped (Figure 2b). When focusing the excitation laser next to the cavity, we observed weak light emission from WSe2 (Fig. 2b, left, dashed circle). This PL emission was significantly enhanced when centering the excitation laser on the NPoM cavity, indicating Purcell enhancement by the plasmonic cavity (Fig. 2b, right). The amplification was tightly localized to the position of the plasmonic nanocavities with up to a 60-fold local increase in PL intensity (Fig. 2c, d). PL spectra from linear scanning across one of the cavities showed that light emission occurs predominantly through the upconverted PL, which has a shorter wavelength than the 785 nm excitation laser (Fig. 2c).
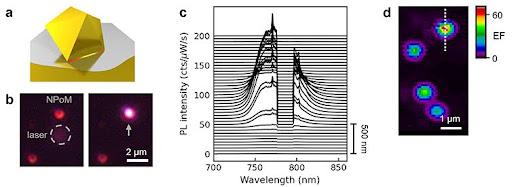
Figure 2: (a) Sketch of a plasmonic cavity with decahedral-shaped nanoparticles (NPoM). The 2D material (grey) defines the nm gap between the nanoparticle and the Au substrate. (b) Dark-field + PL optical image of two NPoM cavities with the excitation laser focused next to the cavity (left) and on the cavity (right). (c) PL spectra from line scanning across the NPoM cavity. The excitation laser lexc = 785 nm is blocked by a notch filter. (d) PL gain map through the four NPoM cavities. The line scan in (c) is indicated by the dashed line. Panels (a), (c) and (d) are taken from Ref. 1.
We explain this strong enhancement of the PL upconversion by the interplay of dark and bright excitons in the WSe2 monolayer. The bright exciton (lbright = 750 nm) has an in-plane transition dipole, while the spin-forbidden dark exciton (ldark = 775 nm) has an out-of-plane transition dipole (Fig. 3a). The plasmonic near-fields of NPoM cavities have both in-plane and out-of-plane electric field components, allowing coupling to both kinds of excitons. This is evident in the Stokes PL spectrum recorded during excitation with a 633 nm laser, i.e., excitation above the WSe2 bandgap, where we observed two emission peaks from the light and dark excitons (Fig. 3b). The reference spectrum recorded next to the cavity showed a weaker PL, dominated by emission from the light exciton (Fig. 3b, gray). This indicates Purcell enhancement of PL emission by the plasmon cavity and activation of PL emission from the dark exciton, which has been previously observed by other groups with plasmon cavities and edge-on approaches 2 3. Using our fully automated setup, we characterized the PL enhancement for 173 NPoM cavities. We found systematically larger PL enhancement of the dark exciton than of the bright exciton (Fig. 3d, compare blue and red). This is due to the fact that the near-plasmonic fields in the nanospace of the NPoM cavity are mostly polarized out-of-plane, leading to preferential coupling to the dark exciton.
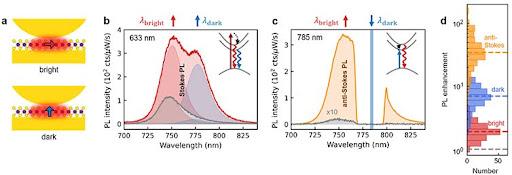
Figure 3 (a) Sketch of the plasmonic coupling to the in-plane transient dipole of the bright exciton (top) and the out-of-plane transient dipole of the spin-forbidden dark exciton (bottom) in the WSe2 monolayer. (b) Stokes PL spectrum (lexc = 633 nm) of the WSe2 monolayer enhanced by the NPoM cavity (red) and recorded next to the NPoM cavity (gray). The two emission peaks come from light (red) and dark (blue) excitons. (c) Anti-Stokes PL spectrum (lexc = 785 nm) enhanced by the NPoM cavity (orange) and next to it (gray). The laser excites the dark exciton and emission occurs from the higher energy bright exciton. See insets in (b) and (c) for energy diagrams. (d) Histogram of PL enhancement factors from measurements on 173 cavities, where the dark and light exciton enhancement is obtained from Stokes emission spectra. Panels (b)-(d) are taken from Ref. 1.
Interestingly, we observed a much larger enhancement of the PL emission when choosing the 785 nm laser excitation (Fig. 3c,d, orange). In this configuration, the excitation laser is close to resonance with the dark exciton, and the light emission is predominantly from the higher energy light exciton (Fig. 3c, anti-Stokes PL). This PL upconversion was almost absent next to plasmonic cavities, indicating that plasmonic cavities activate this process (Fig. 3c). Statistically, we observed a 10- to 100-fold enhancement of PL upconversion, which is an order of magnitude greater than the enhancement of Stokes PL (Fig. 3d, orange). When correlating the enhancement factors, we found that cavities that pair efficiently with the dark exciton also provide a larger PL upconversion. Thus, the dark exciton serves as an excitation channel for PL upconversion, which is activated here by the plasmonic cavities.
Conclusion
In conclusion, we have shown that plasmonic nanoparticles on mirror cavities activate PL upconversion in the WSe2 monolayer. This was achieved through near-field coupling with a dark exciton, which served as an excitation channel, while the emission was from a bright exciton with higher energy. The simple fabrication protocol of plasmonic cavities opens the way to large-scale substrates that may find applications for radiative cooling of 2D materials at the nanoscale, anti-Stokes lasing, and radiative exciton engineering. For these measurements, due to the long wavelength studied, it is important that the system exhibits low dark spot count, low readout noise and high quantum efficiency. This makes the highly configurable Kymera spectrometer and the low-noise Newton EMCCD a suitable choice for these types of experiments.
References
- Mueller et al., Nat. Commun. 14, 5726 (2023), DOI: doi.org/10.1038/s41467-023-41401-8
- Lo et al. Nano Lett. 22, 1915-1921 (2022). DOI: doi.org/10.1021/acs.nanolett.1c04360
- Park et al. Nat. Nanotechnol. 13, 59-64 (2018). DOI: doi.org/10.1038/s41565-017-0003-0